The fast the tortuous: Turn up the heat, and ants turn down the turns
In the recent publication, “Higher soil temperatures cause faster running and more efficient homing in the temperate xerothermophilous ant Formica cinerea (Hymenoptera: Formicidae)”, the authors Piotr Ślipinksi and Xim Cerdá investigate the effect of temperature on the foraging strategy of F. cinerea. In nature, the soil temperature can reach 61 °C, which is above the thermal maximum of most foraging ants. The authors experimentally tested the time and path shape of workers to return to the nest in the field using different temperatures. With increasing temperatures, workers are faster and return in a more direct way to the nest. Here, Theodore (Ted) Pavlic reviews the research and highlights the main points.
A Review by Theodore (Ted) P. Pavlic
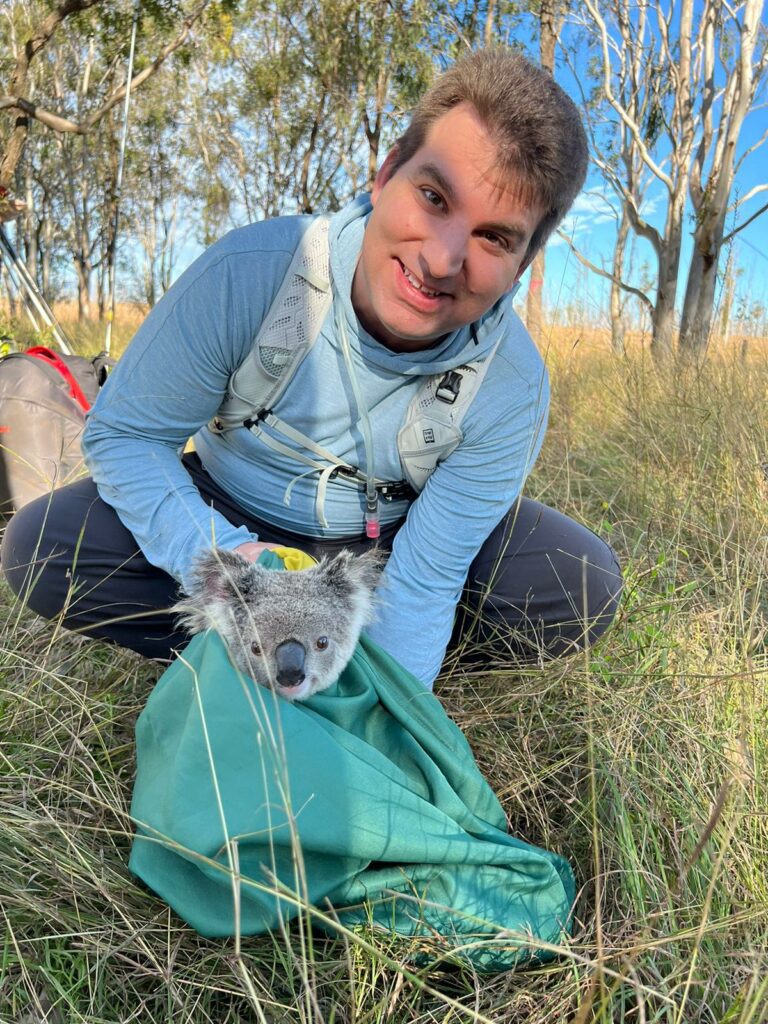
Editors: Biplabendu Das, Phil Hönle, Patrick Krapf
Thermal ecology has historically been a hot topic, and its practical significance only grows over time with concerns about the long-term impacts of climate change on biodiversity. Across animals, temperature plays important roles in development, physiology, and behavior. The adaptation of homeothermy, the ability of an organism to regulate its internal body temperature, in some organisms reduces the conspicuous, observable external variations in performance over short time scales; however, homeothermy comes with more cryptic but significant fitness costs in the long term. For endotherms, regulation of internal body temperature decouples the animal’s ability to operate from the outside, ambient temperature. The metabolic costs of thermal regulation vary with the ambient temperature, and so there is still a connection between environmental temperature and Darwinian fitness for these animals (Levesque and Marshall 2021). Some ectotherms have achieved homeothermy by restricting themselves to habitats with almost no variation in temperature, but this strategy extremely limits access to resources and thus trades temperature costs for other costs. However, most ectotherms are poikilothermic: their internal body temperature varies continuously, and there is usually a very conspicuous relationship between their performance and the external temperature. Furthermore, these animals often have adaptations that allow them to behave differently in different temperatures, like basking in the sun on cool days or adjusting movement patterns on hot days to minimize exposure to the direct sun.
Although some of the best-studied poikilotherms are lizards, ants fall into the poikilothermic category as well. The relationship between environmental temperature and performance in these organisms is often captured by a thermal performance curve (Fig. 1). These curves can be generated empirically by measuring some aspect of performance (e.g., walking speed of an ant, where faster ants are assumed to be at selective advantage relative to slower ants) over a range of different temperatures. Generally speaking, there is a critical lower temperature (CTmin) below which the organism ceases to achieve any appreciable performance and will eventually die if it remains at this temperature for a long period of time (e.g., due to starvation). Then, as temperature is increased above CTmin, the organism’s performance rises until it reaches some maximum and then abruptly crashes until it reaches a critical upper temperature (CTmax) above which it ceases to perform and will eventually die if it remains there for a long period of time (e.g., due to desiccation in very dry environments). Such asymmetry (i.e., slow rise and then abrupt fall) is a common feature of thermal performance curves, which indicates that although poikilothermic organisms will benefit from seeking higher temperatures, they also face an ever-increasing risk of tumbling over a knife edge. Consequently, thermal adaptations should invest the gains from higher temperatures into behaviors that minimize exposure to risks corresponding to life above CTmax.
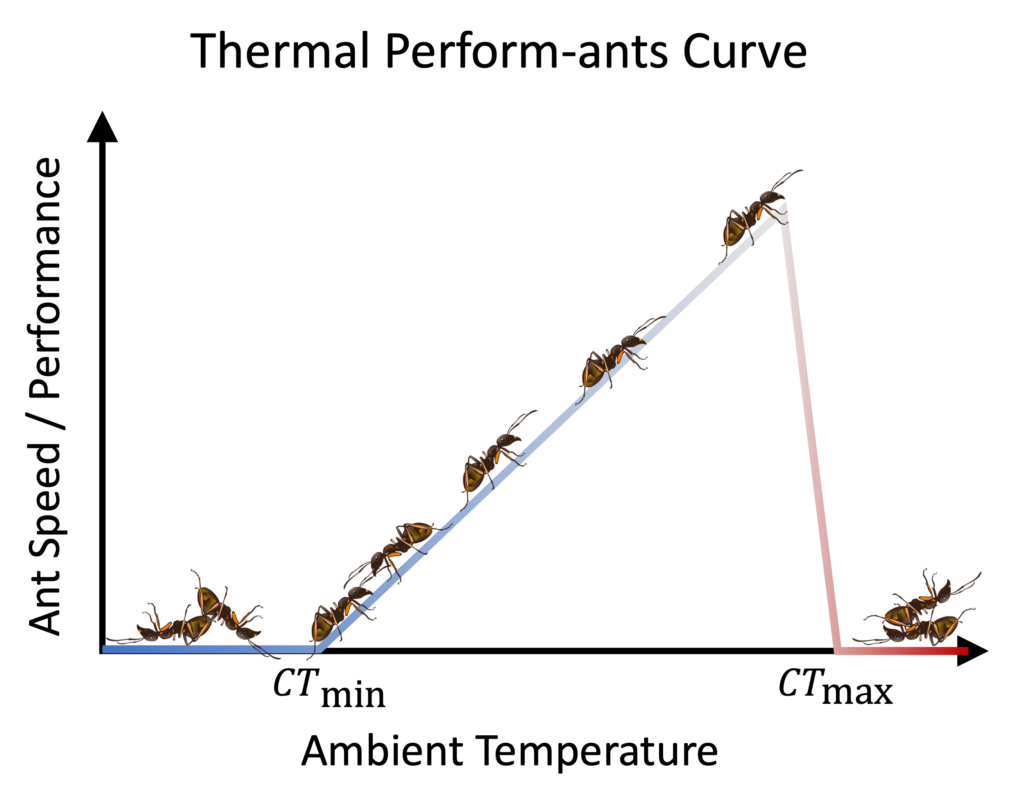
One of the strategies that organisms can use to reduce exposure to high temperatures is to move in paths that are less tortuous. Tortuosity is a measure used to characterize how “curvy” a path is. It has various quantitative definitions, but they are all conceptually similar to the simple definition illustrated in Figure 2, which shows two paths of very different tortuosity. The path on the left has low tortuosity because the total distance moved along the whole path is very close to the straight-line displacement between the start and end of the path. On the contrary, the path on the right has high tortuosity because the path is very long compared to the straight-line displacement between the path’s start and end points. The exposure costs to the tortuous routes like the one on the right might be tolerated by an organism if there is some benefit to this wandering behavior such as greater ability to find scarce resources during foraging. However, at high temperatures (particularly when also dry, as in xeric desert habitats), far less tortuous routes like the one on the left will greatly reduce exposure and thus may be adaptive. One of the first studies of the relationship between temperature, speed, and tortuosity in ants was by Angilletta Jr et al. (2007), who studied tropical leaf-cutter ants whose rainforest environment has a relatively consistent year-round temperature but can have significant temperature variability in microhabitats encountered by the ants. Those authors measured tortuosity in terms of fractal dimension, which is qualitatively similar but quantitatively different than the simple tortuosity expression in Figure 2. They cleverly titled their work, “The fast and the fractalous,” because they uncovered a key tradeoff between ant speed and ant tortuosity – increases in speed (which were related to increases in temperature) led to decreases in tortuosity. They speculated that there could be several biomechanical mechanisms explaining this relationship (e.g., it is harder to make sharp turns at high speeds), but ultimately this pattern could be functional as it might ensure that ants minimize exposure to high-temperature microhabitats by moving quickly while also reducing the curviness of their paths. Since then, Welch et al. (2020) showed that increasing temperature not only increases leaf-cutter ant speed but also increases their intake of leaves. In fact, even the average number of leaves carried per ant increased with temperature, indicating that ants can become more effective at higher temperatures for reasons beyond simply becoming faster. They determined that the critical thermal maximum temperature (CTmax) for these ants were well above the ground temperatures, indicating that slight tropical warming could actually improve herbivory with little risk to these ants. However, the question still remains as to how temperature-related patterns like these would generalize to ants that live in areas with more regular seasonal fluctuations in temperature and maximum temperatures close to or above CTmax.
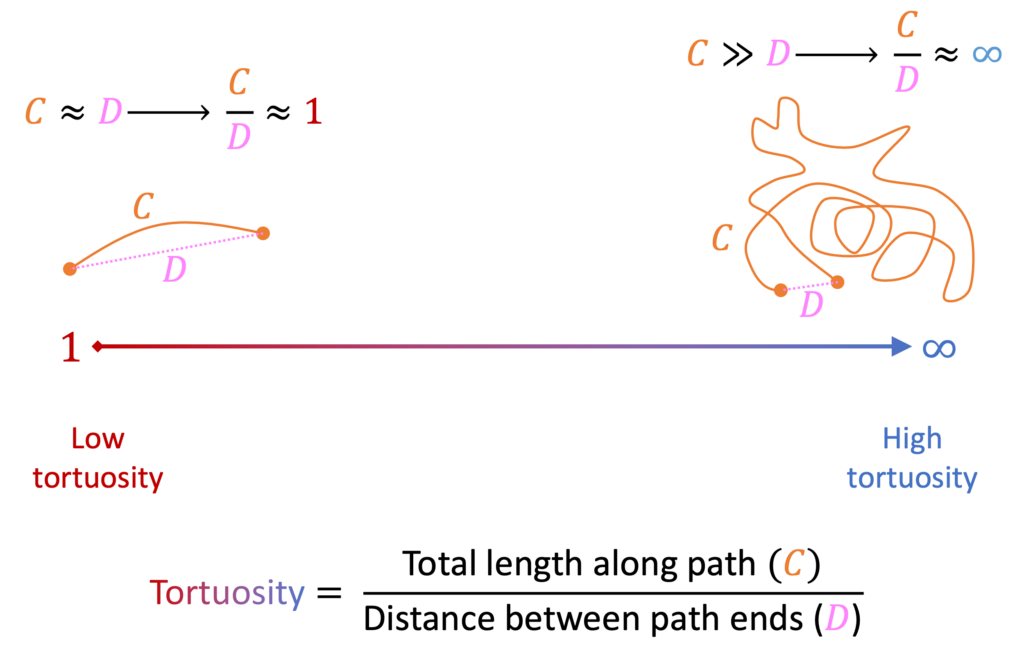
In a paper targeted to swarm-intelligence and engineering communities, Dr. Kaitlin Baudier and I argued that thermoregulation in ants is highly understudied and has the potential to be a rich source for bio-inspired self-organization (Baudier and Pavlic 2022). Our main focus was on the army ants, particularly aboveground species that link their bodies together to form large bivouac structures that serve as temporary homes for the colony while it waits for newly laid eggs to develop into hungry larvae (Figure 3). Whereas ants that make their homes in underground cavities can depend to some extent on buffered temperatures from the earth (a natural form of “geothermal cooling”), ants that evolved to live in aboveground nest structures may need to be more responsive to temperature variations. Despite army ants being poikilothermic, the bivouacs built from their bodies have the ability to regulate an internal temperature that differs from the external temperature (similar to an endotherm), and these bivouacs can also be moved by the colonies to different rainforest regions to find improved humidity and temperature conditions (similar to an ectothermic homeotherm). In other words, aboveground mobile bivouacs are a homeothermic adaptation for army-ant colonies. This army-ant example shows how the thermal ecology of ants is potentially far more complex than the typical poikilothermic model from Figure 1. What other special thermal abilities does colony living afford ants?
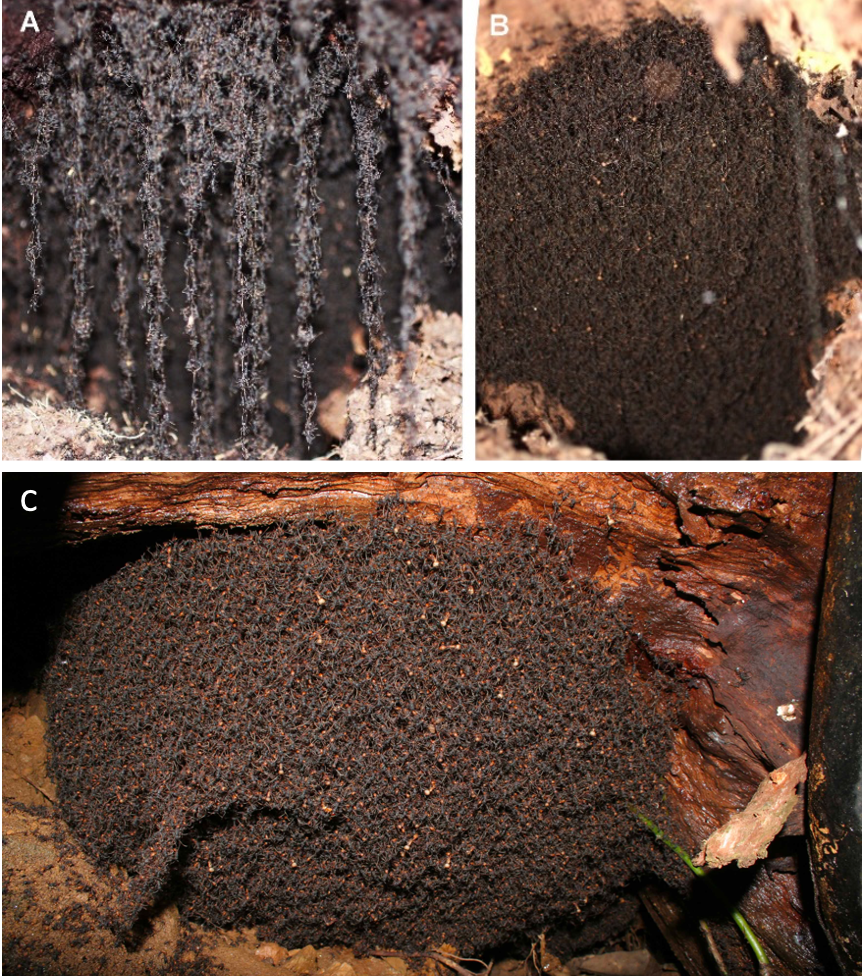
Whereas ants that live in the tropics can depend on high levels of humidity and relatively stable temperatures that are well below their critical thermal maximum, ants adapted for the hot and dry desert likely rely on very different strategies to survive. For example, roughly 200 different species across 50 ant genera have polydomous nests (Debout et al. 2007). That is, instead of inhabiting a single cavity, these ants distribute colony workers, brood, and reproductives across a complex of multiple possibly disconnected cavities. Polydomy provides ants returning to the colony with multiple nest entrances, which together offer multiple exits from potentially unwelcome external conditions (Figure 4). There have been a wide range of proposed explanations for the evolution of polydomy that primarily focus on colony-level performance.
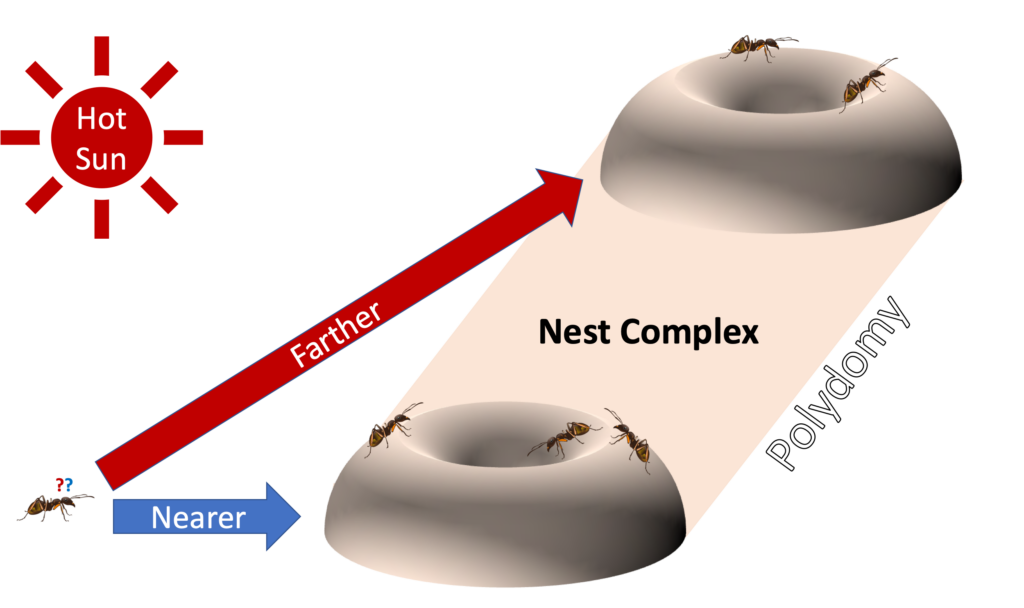
For example, shifting from central-place foraging to central-places foraging can help an ant colony find and secure food from distributed sources (Schmolke 2009) in much the same way content-delivery networks on the Internet ensure that users all over the world can stream the same content despite being far from the ultimate source of that content. Rarely is temperature discussed as a potential driver for polydomy; however, there are a variety of polydomous ants found in the desert with individuals that might thermally benefit from having multiple nest entrances to return to (Figure 4). Two long-legged ants that live in the desert near me, Novomessor cockerelli and Novomessor albisetosus, are not only known for their polydomy but also their particularly good performance at collectively transporting large prey items to one of those available nest entrances (Buffin, Sasaki, and Pratt 2018). Although collective transport in these ants is usually assumed to be defense against mass-recruiting competitors (Markl and Hölldobler 1978), it also seems reasonable that effective cooperative transport like that seen in Novomessor sp. would reduce the amount of time individuals in foraging teams would need to be exposed to hot and dry external conditions. That said, the thermal benefits of polydomy and cooperative transport require one other important property – the ability to move in straight lines (i.e., low path tortuosity) toward nest entrances at high temperatures.
In a new Myrmecological News article from Ślipiński and Cerdá (2022), they investigate the temperature–speed–tortuosity relationships in Formica cinerea. Although it comes from a different subfamily as Novomessor sp., F. cinerea is also a polydomous ant known for its high performance at cooperative transport in sandy, desert environments. This formicine ant is closely related to “wood ants” or “mound ants” that dominate more temperature habitats, and it can similarly dominate its surroundings (unlike the relatively docile myrmicine Novomessor sp.). On one hand, F. cinerea apparently lack the typical adaptations seen in ants that specialize in high-temperature environments. For example, they do not have particularly long legs, and they do not produce protective heat-shock proteins before emerging from their nests. So, it is no surprise that they are found at high latitudes where most thermal specialists are not. However, F. cinerea are also found at low latitudes and have been observed to be active at temperatures normally associated with thermal specialists (54 °C or 129 °F). Consequently, because there are no obvious physiological adaptations that can explain the heat tolerance of individuals of this ant, Ślipiński and Cerdáasked whether there were observable temperature-dependent behavioral changes in this ant that might help explain their high-temperature success.
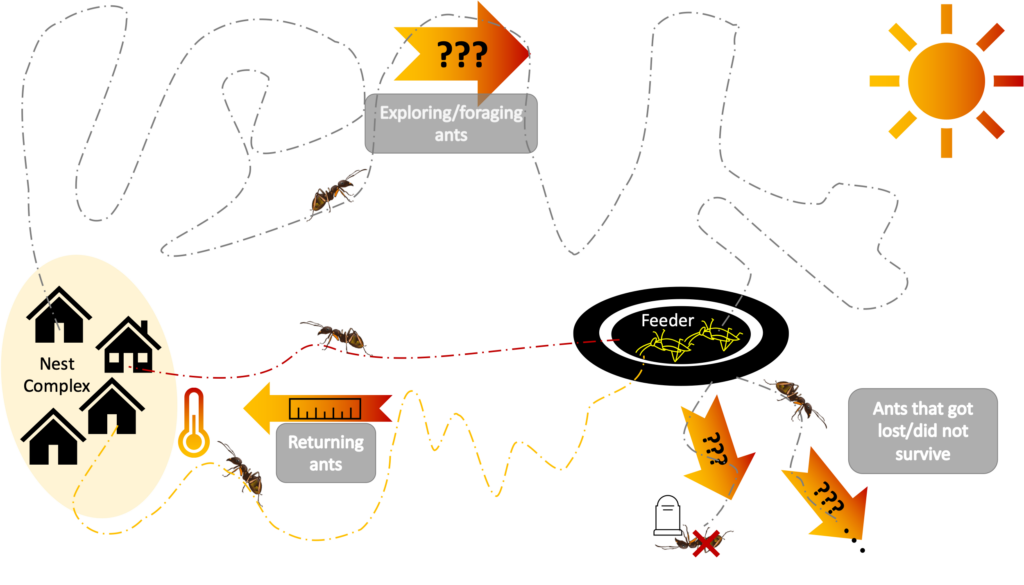
The experimental setup of Ślipiński and Cerdá (2022) is summarized in Figure 5. Multiple nests of Formica cinerea were observed in their natural habitat across a range of temperatures. The study authors provided each ant colony with a large cache of dead fruit flies on a feeder placed relatively close to one of the entrances of each nest complex. Ants were observed: (a) leaving the nest, (b) leaving the feeder but heading in a direction away from the nest, and (c) moving from the feeder back to the nest. Those ants that retrieved a food item from the feeder and traveled back to a nest entrance were video recorded so that their total paths could be analyzed. Additionally, the surface temperature of the sand near those paths were recorded. The authors were then able to study the relationship between surface temperature, ant speed, and path tortuosity (although the authors used a straightness index that is the reciprocal of the tortuosity in Figure 1). Their results were very similar to the results of Angilletta Jr et al. (2007) on leaf-cutter ants: higher temperatures were correlated with higher speeds, and higher speeds were correlated with less tortuosity. In other words, in hot conditions, returning ants spent far less time exposed to hot and dry conditions by being both faster and returning along a more direct path.
Because ants were observed returning along straight paths, Ślipiński and Cerdá (2022) concluded that F. cinerea ants abide by the same speed–tortuosity relationship already found in the literature; faster ants take straighter routes. In their work on leaf-cutter ants, Angilletta Jr et al. (2007) speculated that there might be a biomechanical reason for the speed–tortuosity tradeoff (as opposed to a behavioral reason), just like how faster cars necessarily have larger turning radii. Ślipiński and Cerdá (2022) did not suggest a possible mechanism connecting speed and tortuosity, but they did suggest that ants moving around close to CTmax might suffer heat-related effects that would cause them to become disoriented (which would not occur in the example with leaf-cutter ants). That said, if high temperatures did cause some appreciable fraction of ants to meander aimlessly, it is not clear how this experimental setup would detect it. Ślipiński and Cerdá state that ants moved away from the feeder in different directions, but only ants that returned to an observed nest entrance were included in the analysis. Consequently, there is a possibility that the speed–tortuosity relationship observed by the authors is a result of sampling bias; only the ants that happened to return along a straight path (and thus none of the hypothetical heat-affected ants) would have been included in the statistics. This is not so much a flaw in the study but instead helps highlight that temperature may put a significant selective pressure on these colonies. Although it is possible that selection could shape movement phenotypes so that all ants have a strong speed–tortuosity tradeoff, it is also possible that selection for moving in high temperatures could ensure that there is always some fraction of foragers who do not succumb to the effects of heat.
The other group of F. cinerea ants whose paths were not analyzed by Ślipiński and Cerdá in this study were the outgoing workers, who move in effectively random directions as they search for food. If there truly is a connection between temperature, speed, and tortuosity that causes hot workers to travel high speeds in relatively straight paths, then this might have a negative impact on the search performance of outgoing workers. In other words, meandering in tortuous paths can help an outgoing worker cover more ground. The costs to searching at high speeds may be counterbalanced by the increased speed of movement through the environment, but speed itself often negatively effects the sensory capabilities of predators (e.g., Gendron and Staddon 1983). Consequently, we normally associate meandering paths with exploration for new resources and straight paths with exploitation of discovered resources (Hills et al. 2015; Valentini et al. 2021). Furthermore, ants associated with ephemeral or mobile prey tend to stray from straight paths even when following pheromone trails (Wilson 1962; Dussutour et al. 2009). If a common feature of ant movement is temperature causes tortuosity to decline and speed to increase, then higher temperatures may also be associated with a drop in foraging performance; ants adapted for environments with sparsely distributed or ephemeral prey may be less efficient searchers if forced to operate primarily in hot and dry conditions. This is a biomechanics-mediated information cost of climate change that is not often considered; hot ants may be bad searchers.
In general, there are still many unanswered questions about the role of the environment in the search performance of social-insect groups and other collectives. As exemplified by the thermal movement ecology example from Ślipiński and Cerdá (2022) that motivated this post, understanding the connection between physical variables (e.g., light, temperature, gravity, pressure, and so on) and colony-level, collective performance will require an integrated approach that combines physiology, ecology, and evolutionary biology. In my lab, we have been studying how some of these physical variables affect the tortuosity and speed of teams of another cooperatively transporting ant, the green tree weaver ant Oecophylla smaragdina; stay tuned for that work from Dr. Andrew Burchill (collaborating with Dr. Chris Reid and Dr. Stephen Pratt) in the very near future. That said, we have not yet considered the effect of temperature on cooperatively transporting ants. Comparing the effects of temperature on the cooperative performance of transport teams of Oecophylla smaragdina, Novomessor cockerelli, and Formica cinerea would be an exciting study that I would look forward to reading someday! In the meantime, I hope you take a “bee-line” to Myrmecological News to read the latest from Ślipiński and Cerdá (2022).
References
Angilletta Jr, Michael J., T. C. Roth II, Robbie S. Wilson, Amanda C. Niehaus, and P. L. Ribeiro. 2007. “The Fast and the Fractalous: Speed and Tortuosity Trade off in Running Ants.” – Functional Ecology 22: 78–83. https://doi.org/10.1111/j.1365-2435.2007.01348.x.
Baudier, Kaitlin M., and Theodore P. Pavlic. 2022. “Multi-Level Instrumentation of Bivouac Thermoregulation: Current Methods and Future Directions.” Artificial Life and Robotics, – April. https://doi.org/10.1007/s10015-022-00759-6.
Buffin, Aurélie, Takao Sasaki, and Stephen C. Pratt. 2018. “Scaling of Speed with Group Size in Cooperative Transport by the Ant Novomessor cockerelli.” Edited by Nicolas Chaline. – PLOS ONE 13: e0205400. https://doi.org/10.1371/journal.pone.0205400.
Debout, Gabriel, Bertrand Schatz, Marianne Elias, and Doyle Mckey. 2007. “Polydomy in Ants: What We Know, What We Think We Know, and What Remains to Be Done.” – Biological Journal of the Linnean Society 90: 319–48. https://doi.org/10.1111/j.1095-8312.2007.00728.x.
Dussutour, Audrey, Madeleine Beekman, Stamatios C. Nicolis, and Bernd Meyer. 2009. “Noise Improves Collective Decision-Making by Ants in Dynamic Environments.” – Proceedings of the Royal Society B 276: 4353–61. https://doi.org/10.1098/rspb.2009.1235.
Gendron, Robert P., and John E. R. Staddon. 1983. “Searching for Cryptic Prey: The Effect of Search Rate.” – American Naturalist 121: 172–86.
Hills, Thomas T., Peter M. Todd, David Lazer, A. David Redish, and Iain D. Couzin. 2015. “Exploration versus Exploitation in Space, Mind, and Society.” – Trends in Cognitive Sciences 19: 46–54. https://doi.org/10.1016/j.tics.2014.10.004.
Levesque, Danielle L., and Katie E. Marshall. 2021. “Do Endotherms Have Thermal Performance Curves?” – Journal of Experimental Biology 224: jeb141309. https://doi.org/10.1242/jeb.141309.
Markl, Hubert, and Bert Hölldobler. 1978. “Recruitment and Food-Retrieving Behavior in Novomessor (Formicidae: Hymenoptera): II. Vibration Signals.” – Behavioral Ecology and Sociobiology 4: 183–216. https://doi.org/10.1007/BF00354979.
Schmolke, Amelie. 2009. “Benefits of Dispersed Central-Place Foraging: An Individual-Based Model of a Polydomous Ant Colony.” – American Naturalist 173: 772–78. https://doi.org/10.1086/598493.
Valentini, Gabriele, Theodore P Pavlic, Sara Imari Walker, Stephen C Pratt, Dora Biro, and Takao Sasaki. 2021. “Naïve Individuals Promote Collective Exploration in Homing Pigeons.” – ELife 10: e68653. https://doi.org/10.7554/eLife.68653.
Welch, L. E., Kaitlin M. Baudier, and Jon F. Harrison. 2020. “Warmer Mid-Day Temperatures Increase Leaf Intake by Increasing Forager Speed and Success in Atta colombica during the Rainy Season.” – Insectes Sociaux 67: 213–19. https://doi.org/10.1007/s00040-020-00749-6.
Wilson, Edward O. 1962. “Chemical Communication among Workers of the Fire Ant Solenopsis saevissima (Fr. Smith): 2. An Information Analysis of the Odour Trail.” – Animal Behaviour 10: 148–58. https://doi.org/10.1016/0003-3472(62)90142-2.
Recent Comments